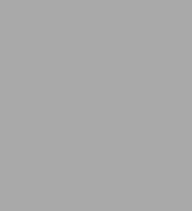
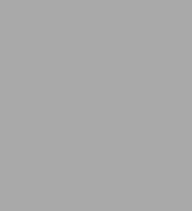
eBook
Related collections and offers
Overview
This volume, which employs a relatively simple approach in order to reach the widest audience, focuses on two main topics: classical light scattering (scattering intensity, concentration dependence, size dependence, and polydispersity) and dynamic light scattering (time and frequency dependence, translational diffusion, directed flow, rotational motion, and more). A series of useful appendixes and a list of references complete this concise, accessible work, a valuable resource for physicists, chemists, and anyone interested in the increasingly important field of laser light scattering.
Product Details
ISBN-13: | 9780486152202 |
---|---|
Publisher: | Dover Publications |
Publication date: | 03/02/2015 |
Series: | Dover Books on Physics |
Sold by: | Barnes & Noble |
Format: | eBook |
Pages: | 112 |
File size: | 5 MB |
Read an Excerpt
Laser Light Scattering
By Charles S. Johnson Jr., Don A. Gabriel
Dover Publications, Inc.
Copyright © 1981 CRC Press, Inc.All rights reserved.
ISBN: 978-0-486-15220-2
CHAPTER 1
INTRODUCTION
Light scattering has provided an important method for characterizing macromolecules for at least three decades. However, the replacement of conventional light sources by lasers in recent years has qualitatively changed the field and has sparked renewed interest. Through the use of intense, coherent laser light and efficient spectrum analyzers and autocorrelators, experiments in the frequency and time domains can now be used to study molecular motion, e.g., diffusion and flow, and other dynamic processes, as well as the equilibrium properties of solutions. The technology for clarifying samples has also significantly improved. Recirculation through submicron filters in closed loop systems reduces the effects of dust and other contaminants, and the new time domain techniques provide built-in tests for the presence of such particles. These advances make laser light scattering a powerful form of spectroscopy for use in biochemistry.
Classical light scattering studies are concerned with the measurement of the intensity of scattered light as a function of the scattering angle. In addition to this kind of study, laser light sources now permit spectral information to be obtained from the scattered light. The latter type of experiment is often called quasi-elastic light scattering (QLS), and the various forms of the experiment are known as light beating spectroscopy (LBS), intensity fluctuation spectroscopy (IFS), and photon correlation spectroscopy (PCS). Related experiments in laser doppler velocimetry (LDV) now permit very low rates of uniform motion to be measured. A special case of LDV is electrophoretic light scattering (ELS) where mobilities are determined. The aim of this book is to provide an introduction to both the classical and quasi-elastic forms of laser light scattering, which can serve as an introductory text for students and a reference for research workers. The same purpose has been served for prelaser classical light scattering since 1960 by Chapter 5 of Tanford's excellent book, Physical Chemistry of Macromolecules. As in that chapter, we emphasize concepts and the kinds of information that can be obtained from the various experiments rather than either presenting a comprehensive survey of the literature or discussing experimental techniques in great detail. For the most part we stick to well- developed techniques such as the measurement of translational and rotational diffusion coefficients. A few specialized applications (such as the study of motility) are also discussed; however, we have not included scattering from internal modes in polymers or gels. Interesting work is going on in the latter areas, but the theory is still in flux.
We attempt to keep the objectives in sight at all times and to avoid distracting complications as much as possible. Accordingly, certain definitions and derivations have been relegated to a set of appendixes. In general, we have opted for simplicity rather than elegance, or even rigor in certain cases, in the hope that a wider audience can be reached. For those who desire more extensive treatments there is an abundance of sources. For classical scattering, one of the latest reviews is that by Timasheff and Townsend, which is concerned with proteins. Also Huglin has edited an extensive volume that emphasizes experimental methods and data handling in the study of polymer solutions. The book by Fabelinskii is a comprehensive, but difficult treatment mainly of prelaser light scattering without special attention to macromolecules. The classical physics of light scattering has been treated in detail by Kerker. The book by Long, while emphasizing Raman scattering, provides a good introduction to Rayleigh scattering, especially polarization and electronic resonance effects. Quasi-elastic scattering, also called dynamic light scattering, has received considerable attention in the literature, and the recent review papers are too numerous to list in full. An encyclopedic review of dynamic light scattering from biopolymers and biocolloids has been provided by Schurr. Pusey and Vaughan have given a much briefer review of the principles of intensity fluctuation spectroscopy, and Carlson has covered applications in molecular biology. Laser velocimetry has been reviewed by Ware. An introduction to the theoretical foundations of dynamic light scattering is presented in the book by Berne and Pecora, which is an excellent text for students of physical chemistry. The book by Chuis a good reference for many experimental and theoretical details. For those attempting to understand photon statistics and photon correlation spectroscopy, the monograph edited by Cummins and Pike is invaluable.
CHAPTER 2CLASSICAL LIGHT SCATTERING
A. Scattering Intensity
1. Scattering by an Isolated Dipole and by Gases
Lasers produce collimated, quasi-monochromatic radiation having high intensity. In all but the least expensive lasers, the output is highly polarized. For discussion of the scattering experiment we adopt the coordinate system shown in Figure 1, where the incident laser light propagates in the + y-direction, and the x and y axes define the scattering plane. Only the electric field of the incident light is of interest here, and we assume that the incident light is polarized so that the electric field is in the z-direction. We express the electric field of the incident light as
[MATHEMATICAL EXPRESSION NOT REPRODUCIBLE IN ASCII] (1)
where ko = 2π/λo, λo is the wavelength in vacuum, and ωo = 2πvo is the laser frequency. The electric field E, interacts with electrons in an atom or molecule to induce an electric dipole moment, which oscillates at the angular frequency ωo. The usual expression for the induced dipole is
[MATHEMATICAL EXPRESSION NOT REPRODUCIBLE IN ASCII] (2)
where in general is a vector not necessarily in the same direction as [??] and [??] is the polarizability tensor that relates the two vectors. A discussion of the polarizability tensor is given in Appendix A. We shall mainly be concerned with isotropic scatterers for which [??] is independent of orientation and Equation 2 becomes
[MATHEMATICAL EXPRESSION NOT REPRODUCIBLE IN ASCII] (3)
where α is a constant.
It is well known in electromagnetic theory that an oscillating dipole produces radiation — as for example with the oscillating currents in antennas of radio transmitters. The oscillating electric moment pz thus provides the source of the scattered light. This is equivalent to the statement that an accelerating charge generates electromagnetic radiation. The solution of Maxwell's equations for an oscillating dipole shows that at large distances, i.e., where R >> λo, the electric field E, of the scattered light is proportional to d2p/dt2 = -[??]p and inversely proportional to distance R as required by the conservation of energy. The complete expression in SI units for the field E, at R resulting from dipole at the origin in Figure 1 is
[MATHEMATICAL EXPRESSION NOT REPRODUCIBLE IN ASCII] (4)
where χ is the angle between the induced moment p and [MATHEMATICAL EXPRESSION NOT REPRODUCIBLE IN ASCII]is the permittivity of free space, and c is the speed of light. When the detector is in the xy plane, the factor sinχ is of course unity.
Detectors, e.g., photomultiplier tubes, respond to the intensity rather than the electric field of incident light. The instantaneous intensity, which is defined as the rate of passage of energy through unit area perpendicular to the direction of propagation, is given by
I = ceo E2(5)
Since all measurements require times that are much larger than the oscillation period of the radiation field, we are usually concerned with the cycle average of I. Thus for the incident radiation
[MATHEMATICAL EXPRESSION NOT REPRODUCIBLE IN ASCII] (6)
In calculations involving electric fields it is often convenient to use complex variables, e.g.,
[MATHEMATICAL EXPRESSION NOT REPRODUCIBLE IN ASCII]
rather than [MATHEMATICAL EXPRESSION NOT REPRODUCIBLE IN ASCII] When the complex variables are handled properly, derivations are simplified, but the final results are not changed. For example, if the electric field of the incident radiation is written in complex form, the cycle average of the intensity can be written as
[MATHEMATICAL EXPRESSION NOT REPRODUCIBLE IN ASCII] (7)
where Re means "the real part of" and E* is the complex conjugate of E.
Using Equation 6 the intensity of the scattered light is given by [MATHEMATICAL EXPRESSION NOT REPRODUCIBLE IN ASCII], and Equation 4 yields
[MATHEMATICAL EXPRESSION NOT REPRODUCIBLE IN ASCII] (8)
From Equations 3 and 6 we see that [MATHEMATICAL EXPRESSION NOT REPRODUCIBLE IN ASCII], and Equation 8 gives
[MATHEMATICAL EXPRESSION NOT REPRODUCIBLE IN ASCII] (9)
The inverse fourth power dependence on λo was predicted by Lord Rayleigh on the basis of simple dimensional arguments. It accounts for the blue color of the sky, since molecules in the atmosphere tend to scatter the blue part of the solar spectrum with greater intensity than the longer wavelength red components. At optical frequencies almost all of the scattering results from electrons, and the number of electrons increases with molecular volume. Therefore, the polarizability is roughly proportional to molecular volume, especially for larger molecules, and the intensity accordingly depends on the square of the volume. In Equation 9 and in other parts of this book we have used SI units, which are based on the MKS system, except when established definitions were preserved in cgs units. The equations can be converted to the cgs system simply by replacing 4πεo with unity.
The intensity of scattering per unit volume for a gas at low pressure can be obtained by multiplying Equation 9 by N, the number of scatterers per unit volume. It is conventional, however, to rewrite this equation in terms of the change of refractive index with concentration, which is the experimentally determined quantity, rather than the molecular polarizability. If C is the mass per unit volume of the gas, then at low pressures the refractive index n can be expanded in a Taylor's series
[MATHEMATICAL EXPRESSION NOT REPRODUCIBLE IN ASCII] (10)
so that
[MATHEMATICAL EXPRESSION NOT REPRODUCIBLE IN ASCII] (11)
However, as discussed in Appendix B, n2 is also given by
n2 = 1 + na/eo(12)
The combination of Equations 11 and 12 permits us to write
[MATHEMATICAL EXPRESSION NOT REPRODUCIBLE IN ASCII] (13)
where m is the mass per scattering particle. To obtain the intensity of scattering per unit volume, we substitute Equation 13 into Equation 9 and multiply by N = NAC/M where NA is Avogadro's number and M is the molecular weight. Thus
[MATHEMATICAL EXPRESSION NOT REPRODUCIBLE IN ASCII] (14)
Scattering experiments are usually reported in terms of the Rayleigh ratio Rθ defined by
[MATHEMATICAL EXPRESSION NOT REPRODUCIBLE IN ASCII] (15)
where I, is the measured intensity at θ,, V is the volume of the scattering region, and the bars on I, and I, have been dropped, as they will be henceforth, for convenience. Equation 15 is not very useful in practice, since the radiant power P rather than the intensity I is usually measured by photomultiplier tubes and the scattering volume V is not known with accuracy. A more practical expression for [??]θ, is shown in Equation 16.
[MATHEMATICAL EXPRESSION NOT REPRODUCIBLE IN ASCII] (16)
Here P, is the radiant power of the light collected at the scattering angle θ, P, is the radiant power of the incident beam, ΔΩ is the solid angle of the scattered light that is collected, and I is the length of the scattering volume. The conversion of Equation 15 into Equation 16 proceeds by using the cross-sectional areas A, and A, = R2(ΔΩ) of the incident and scattered beam, respectively, where R is the distance from the scattering volume to the detector, with the definitions Iθ = Pθ/Aθ, I, = Pi/Ai, and V = l A,.
One additional comment about Equation 14 is in order before we proceed to the consideration of condensed phases. We have assumed that the incident light is polarized (along the z-direction) and that the scattering particles have dimensions much smaller than the wavelength of the incident light. As a consequence, the intensity is independent of the scattering angle θs. However, if either (1) the incident light is unpolarized, or (2) all of the scattered light in the cone θs to θs, + dθs is collected regardless of the angle χ, then the factor sin χ must be replaced by [MATHEMATICAL EXPRESSION NOT REPRODUCIBLE IN ASCII]. This factor is obtained by averaging sin2χ over the angle γ. By inspection of Figure 1 it is seen that cosχ = sin θ2 cos γ. Therefore, [MATHEMATICAL EXPRESSION NOT REPRODUCIBLE IN ASCII] and
[MATHEMATICAL EXPRESSION NOT REPRODUCIBLE IN ASCII] (17)
In practice we find that either χ is set at 90° or that light is collected at all values of χ. In the following χ is assumed to be 90° unless otherwise stated.
2. Scattering by Macromolecules in Solution
In contrast to light scattered from gases, the intensity of scattered light from condensed phases is less than that predicted by Equations 9 and 14. The reduced intensity is the result of destructive interference. In fact, for perfect crystals irradiated with light, the wavelength of which is much greater than the separation of the lattice planes, no light is scattered. This follows from the fact that it is always possible to pair two scattering planes so that destructive interference occurs. Scattering from crystals is possible at certain angles, however, when the wavelength of the incident radiation is roughly equal, to the distance d separating the scattering planes. The condition for scattering is the well-known Bragg relation, sin (θ2/2) = nλ/2d where θ2 is defined in Figure 1.
On the other hand, the scattering centers in liquids are not stationary, but undergo Brownian movement, which produces transient optical inhomogenities in the solution.
It is the presence of these inhomogenities that allows a small fraction of the scattered radiation to escape destructive interference and be observed as scattered intensity outside the sample. Our task is to formulate a theory that relates the intensity of scattered light to these transient optical inhomogenities. The basic ideas in the fluctuation theory of light scattering are attributed to Smoluchowski and Einstein. The problem can be approached by considering the solution of scatters as being composed of N small volume elements per unit volume. The volume elements dV are assumed to be small relative to the wavelength of the incident radiation. The light scattered from the independent volume elements largely cancels, but at any instant there will be a deviation from the time average number of particles in any volume element, and the cancellation will be incomplete. The connection with the scattering theory developed in Section II.A.1 is made by realizing that fluctuations in concentration or density lead to fluctuations in the polarizability. The fluctuation in the polarizability of one volume element is defined as [MATHEMATICAL EXPRESSION NOT REPRODUCIBLE IN ASCII] where αv is the instantaneous polarizability and [bar.α]v is the time average of αv,. A fluctuation can obviously be either positive or negative, and from the definition the time average of δαv. is zero. In Equation 9 the intensity of the scattered radiation is shown to be proportional to the square of the polarizability. To obtain the contribution from an average volume element, we square the quantity, αv = [bar.α]v + δαv, and take the time average. Thus,
[MATHEMATICAL EXPRESSION NOT REPRODUCIBLE IN ASCII] (18)
The contribution from ([bar.α]v)2 cancels exactly as in perfect crystals, and the net scattering intensity depends on [MATHEMATICAL EXPRESSION NOT REPRODUCIBLE IN ASCII]. Substituting this result into Equation 9 and multiplying by N = 1/δV gives
[MATHEMATICAL EXPRESSION NOT REPRODUCIBLE IN ASCII] (19)
for the scattering intensity per unit volume. To obtain [MATHEMATICAL EXPRESSION NOT REPRODUCIBLE IN ASCII] in terms of more readily measurable experimental quantities, we note that the mean square fluctuation in the polarization for a given volume element is related to the mean square fluctuation in concentration by
[MATHEMATICAL EXPRESSION NOT REPRODUCIBLE IN ASCII] (20)
The smaller contributions resulting from temperature and volume fluctuations are neglected in this derivation, since these contributions are expected to be the same for the solution as for the solvent. It is expected that the experimental measurements can be corrected so that only the "excess" scattering by the solute is obtained.
In Appendix B it is shown that the polarizability is related to the refractive index of the solution by
[MATHEMATICAL EXPRESSION NOT REPRODUCIBLE IN ASCII] (21)
This equation can be differentiated with respect to the concentration to obtain an expression for ([partial derivative]α/[partial derivative]C)[??] v in terms of the measurable quantity ([partial derivative]α/[partial derivative]C)τ, v. Thus
[MATHEMATICAL EXPRESSION NOT REPRODUCIBLE IN ASCII] (22)
The relationship between the mean-square fluctuation in the polarizability and the mean-square fluctuation in the concentration is obtained by combining Equations 20 and 22.
(Continues...)
Excerpted from Laser Light Scattering by Charles S. Johnson Jr., Don A. Gabriel. Copyright © 1981 CRC Press, Inc.. Excerpted by permission of Dover Publications, Inc..
All rights reserved. No part of this excerpt may be reproduced or reprinted without permission in writing from the publisher.
Excerpts are provided by Dial-A-Book Inc. solely for the personal use of visitors to this web site.
Table of Contents
Contents
DOVER BOOKS ON PHYSICS,Title Page,
Copyright Page,
PREFACE,
I. INTRODUCTION,
II. CLASSICAL LIGHT SCATTERING,
III. DYNAMIC LIGHT SCATTERING,
APPENDIXES,
REFERENCES,